پوشش نانو؛
نازک اما به استحکام فولاد

پرسنل آموزش دیده و متخصص

۲۵۰+ پروژه جابهجایی دفتر کار

ارائه گارانتی سلامت کالا
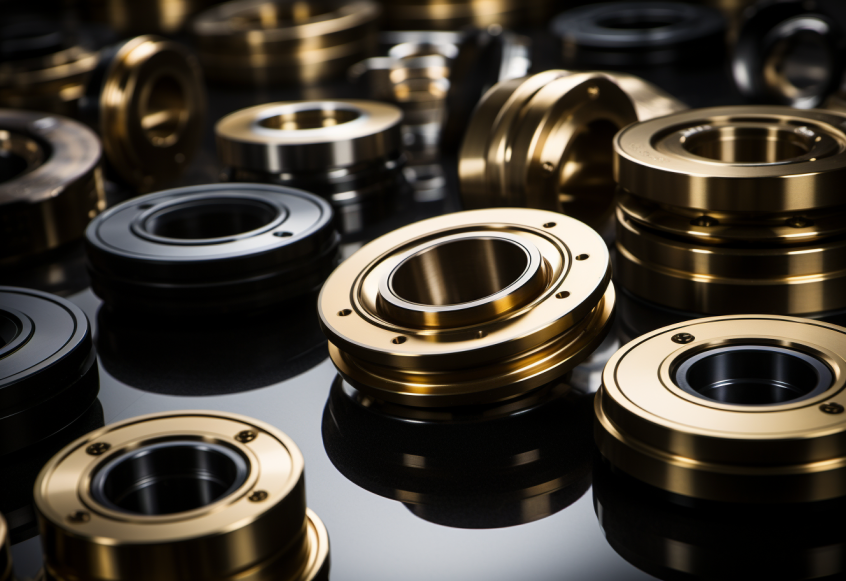
چشمه نور در سال ۱۳۹۷، دریچهای نو در صنعت روشنایی گشود. این صنعتگر قدیمی با بهرهگیری از فناوری نانو و دستگاههای پیشرفته، در زمینه آبکاری لوسترهای فلزی به خودکفایی رسید. این تحول شگفتانگیز، نه تنها به گسترش فعالیتهای چشمه نور انجامید، بلکه آن را به قطب نوآوری در صنعت روشنایی تبدیل کرد. در سال ۱۳۹۸، با تکیه بر سرمایه انسانی خلاق و تجهیزات مدرن، نانو پلاس گامی فراتر نهاد و خدمات خود را به برندهای بزرگ کشور گسترش داد. چشمه نور در طی این سالها آزمایشگاه نانوپلاس خود را گسترش و بهروزرسانی نموده و با قدرت هرچه تمامتر به مسیر پیشرفت ادامه میدهد.
ارتباط با نانوپلاس
جدیدترین های نانوپلاس
۳۰
دی
معرفی مرکز آبکاری نانو در تهران و کرج
مرکز آبکاری نانو در تهران، البرز و کرج: آبکاری نانو چیست؟ چرا وسایل را آبکاری نانو میکنند؟ و مرکز آبکاری نانو چه ویژگیهایی دارد؟ در ...
۰۸
دی
مرکز آبکاری فلز کجاست؟
اگر به دنبال بهترین مرکز آبکاری فلز هستید، ادامه این مطلب را از دست ندهید. آبکاری وسایل فلزی به دلایل گوناگونی همچون جلوگیری از آسیب و ...
۰۸
دی
آبکاری لوستر در تهران
معرفی آبکاری لوستر در تهران : یکی از مهم ترین ویژگی های لوستر ها، زیبایی و درخشندگی آنها است. به این ترتیب برای این که بدنه لوسترهای فل...
۱۱
آذر
همه چیز درباره آبکاری فلزات
آبکاری فلزات چیست؟
آبکاری فلزات در واقع لایه نازکی از فلز است که به قسمت بیرونی یک ماده اضافه شده است. فرایند آبکاری فلزات یک فرآیند پ...
۱۰
آذر
آشنایی با ۳ مزیت آبکاری لوستر
مزیت آبکاری لوستر: به نظر شما لوسترهای آبکاری شده نسبت به لوسترهای دیگر چه مزایایی دارند؟ همانطور که می دانید، بدنه لوسترهای فلزی پس از...
۰۸
آذر
مزیت آبکاری نانو نسبت به سایر روش ها
بررسی مزایای آبکاری نانو
مزیت آبکاری نانو بسیار گسترده است. برای چندین دهه تولیدکنندگان به دنبال راه حلی درخشش طولانی مدت بوده اند که ...
۰۶
آذر
آشنایی با انواع روش آبکاری استیل
استیل نوعی فولاد مقاوم است که در برابر زنگ زدگی و خوردگی شیمیایی مقاومت زیادی دارد. در این مقاله از وبلاگ نانوپلاس پیش از آن که روش آب...
۰۴
آذر
انواع روش آبکاری نانو
تاریخچه آبکاری
پیش از اینکه درباره روش آبکاری نانو صحبت کنیم اجازه بدهید که نگاهی به تاریخچه آبکاری بیندازیم. پیش از این داستان آبکاری...